Birch Reduction
In this tutorial, I want to talk about the Birch reduction, which is an interesting aromatic reaction that converts an aromatic compound into a 1,4-cyclohexadiene by reducing the aromatic ring and destroying its aromaticity in the process. One fascinating aspect of this reaction is that we can get two possible variations of our product: one where the double bonds are located next to the substituent and another where the double bonds are aligned away from it. This regioselectivity depends on the nature of the substituents. But to really understand why this happens, we need to look at the mechanism.
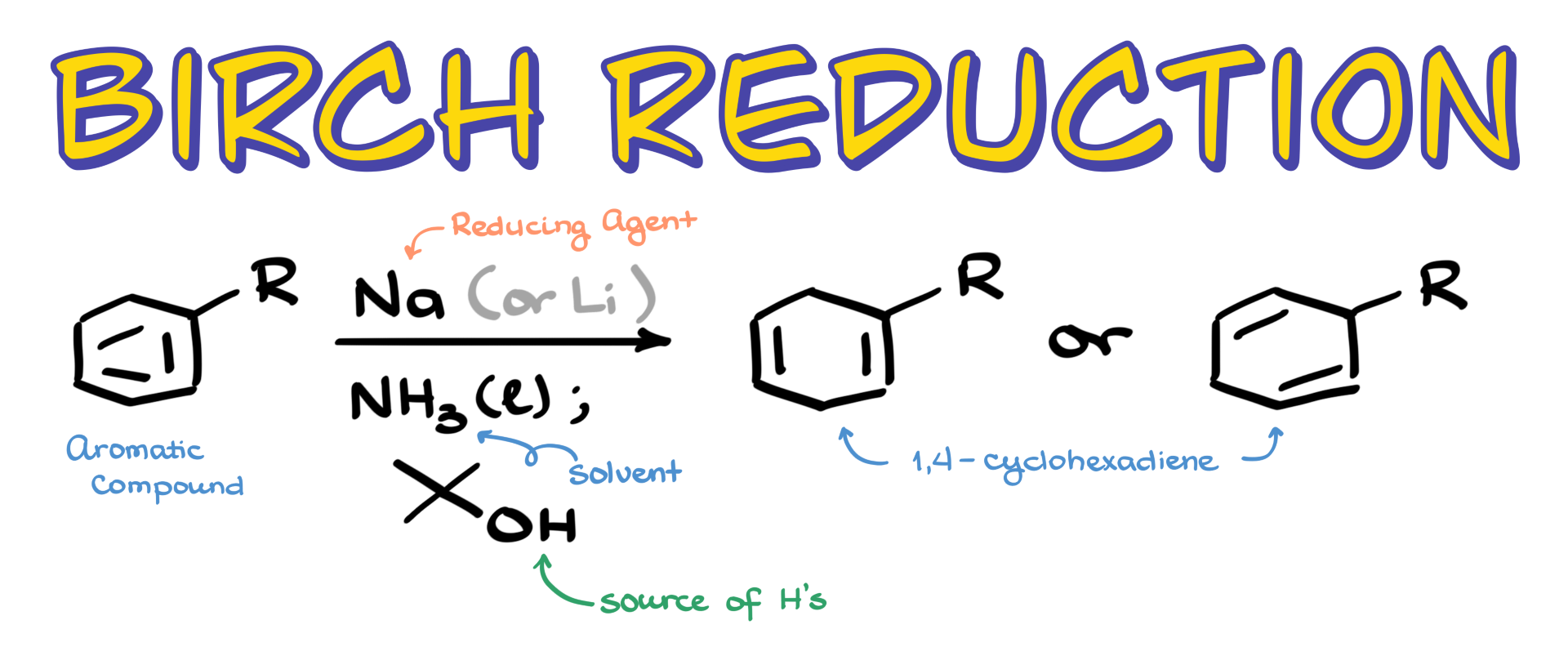
Mechanism of the Birch Reaction
Let’s start with the simplest case possible: the reaction of benzene with sodium and liquid ammonia in the presence of an alcohol. Now, while I’m not going to be showing ammonia explicitly in the mechanism, it’s absolutely essential for this reaction. What happens is sodium (Na) sheds its electrons, and those electrons get solvated in the liquid ammonia. Without liquid ammonia as the solvent, the reaction just won’t work. I’m going to represent these solvated electrons as e⁻. Some textbooks and instructors show this as sodium with an electron, which is technically incorrect, so I won’t be using that notation.
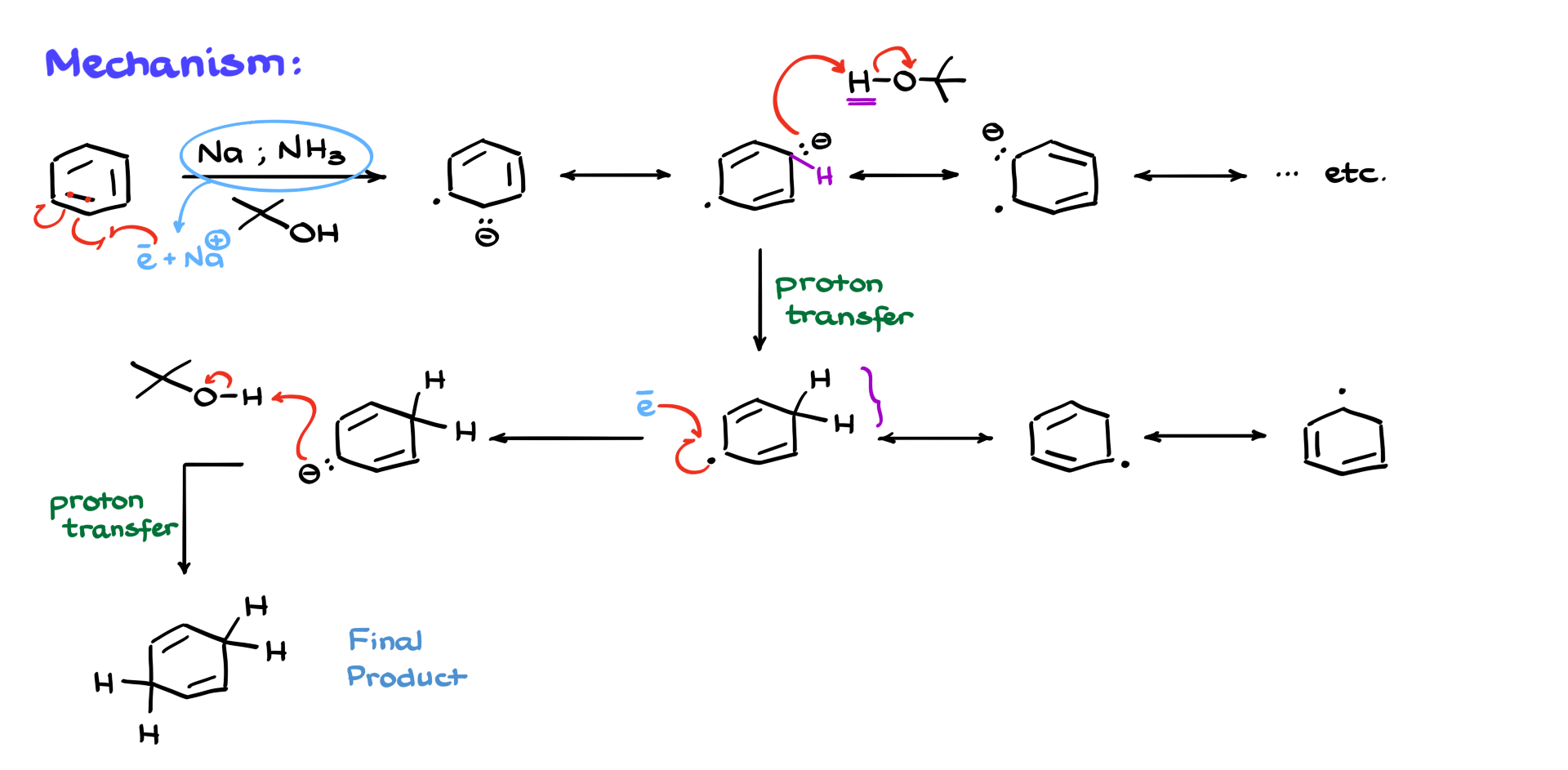
Since sodium ultimately just becomes a positively charged counterion, I’m not going to mention it much anymore. The first step in the mechanism involves an electron interacting with our aromatic ring. We’ll take a double bond in the ring, and one electron from that bond will interact with our solvated electron, while the other stays on a carbon of the aromatic ring. This gives us a somewhat exotic structure with an ion and a radical, but you’ll get used to seeing these kinds of intermediates throughout this tutorial. These are called an anion radicals.
From here, we can draw several resonance structures. I’ll show just a few, but there are plenty you can explore. The key point to remember is that the structure where the anion and the radical are far apart is typically the major contributor. This is because when those species are next to each other, both computational and experimental studies show that the intermediate becomes much less stable.
Moving forward with the mechanism, we now bring in a source of protons—our alcohol. Usually, this is tert-butanol, although methanol, ethanol, regular butanol, and other alcohols can also be used. But the classic example uses tert-butanol, so that’s what I’ll go with here. The alcohol protonates the anionic part of our molecule, giving us a new intermediate. Now we have an sp³-hybridized atom with two hydrogens: one from the original molecule and one from the alcohol. We can draw a couple of resonance structures for this intermediate, but not as many as before.
Next, our radical reacts with another solvated electron, recombining to form another anionic species. That anion is then protonated by another equivalent of the alcohol, giving us the final product—a 1,4-cyclohexadiene. While we could theoretically draw resonance structures that show the negative charge in different positions, experimentally we see that the product is overwhelmingly the 1,4-diene. The deeper explanation for this lies in molecular orbital theory, which is beyond the scope of this tutorial, but rest assured there’s a solid reason for it.
That was the simplest example. Now, what happens when we introduce substituents?
Substituent Effects in the Birch Reduction
Let’s take anisole as an example, which has a methoxy group on the aromatic ring. In this case, we could get two possible products, but spoiler alert—it’s going to be the second option. Let’s walk through the mechanism to understand why.
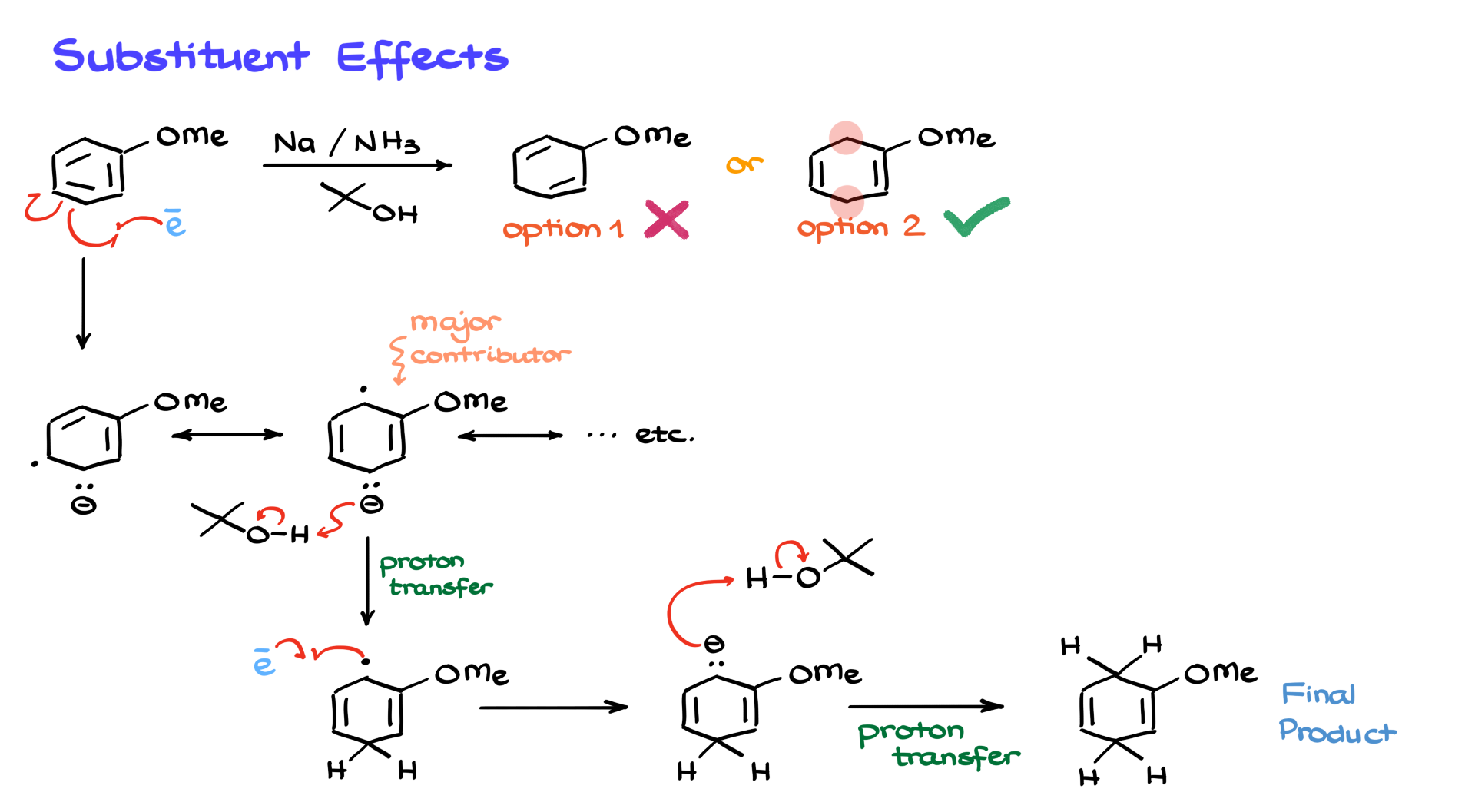
As before, a solvated electron interacts with the aromatic ring, generating our intermediate. Again, we’ll focus on the major resonance contributor where the anion and the radical are far apart. While the negative charge and the radical could theoretically end up on any carbon in the ring, the most stable arrangement places them where they are as far from each other (and from other electron pairs) as possible. Electron pairs sitting too close to each other create unfavorable electrostatic interactions.
Once we have our major contributor, the anionic part grabs a proton from tert-butanol, forming a new radical intermediate. Then, another solvated electron comes in and reacts with the radical, giving us another anion. We protonate this again with tert-butanol, forming our final product. When you have an electron-donating group like a methoxy group, the sp³-hybridized atoms (where the former double bonds were reduced) end up away from the group. This makes sense because you don’t want a negative charge sitting next to an electron-donating group.
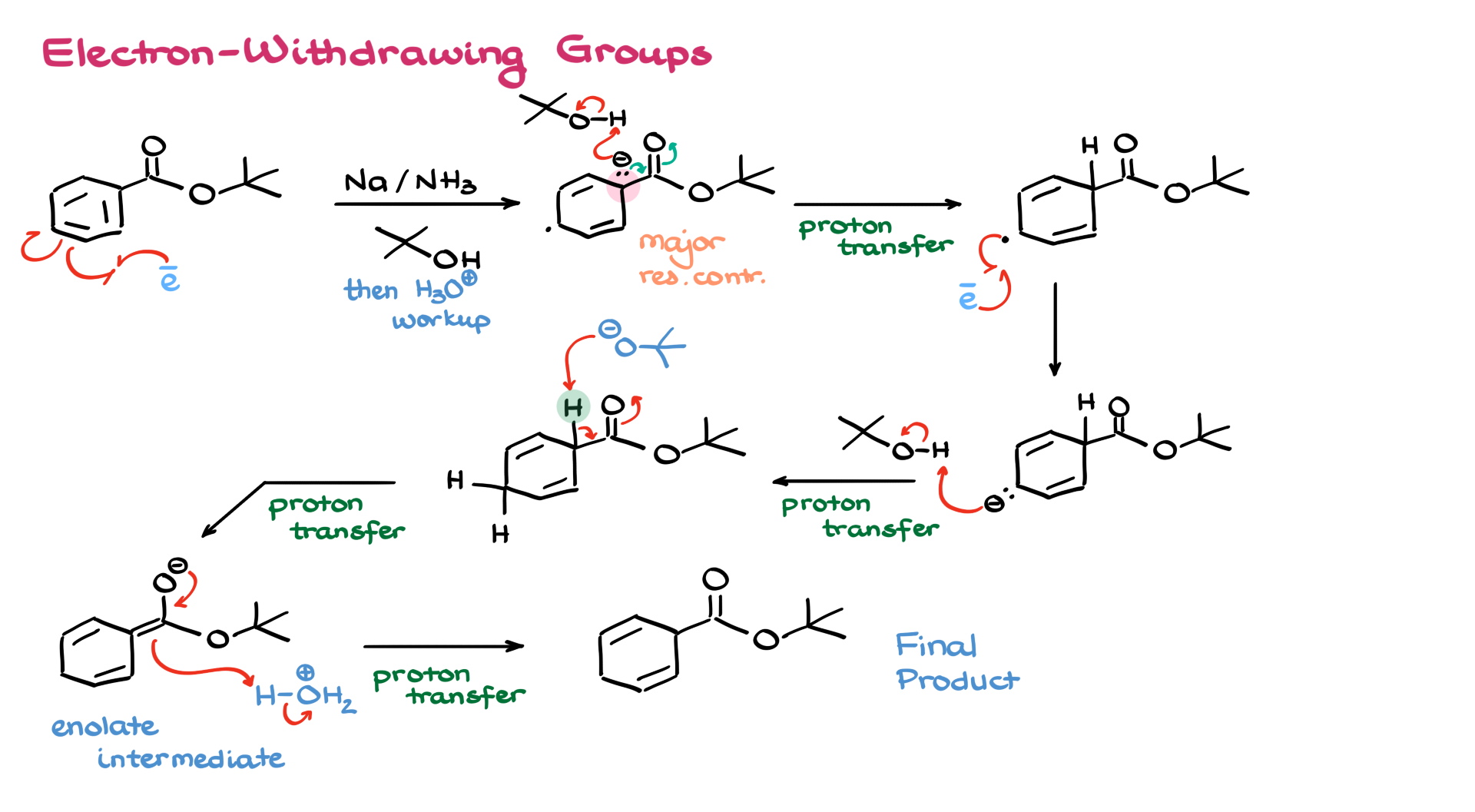
Now, what about an electron-withdrawing group? Let’s look at that. Once again, we bring in our electron, form the radical anion, and immediately look at the major contributor. In this case, the negative charge is stabilized by the electron-withdrawing group. We can even draw a resonance structure that places the charge right into the group itself. From here, the anion grabs a proton from tert-butanol, and then another electron is added to give the final anionic intermediate. A second protonation gives us the product.
Here’s where it gets interesting. The product now contains a hydrogen between the withdrawing group and a double bond—an acidic position. So our tert-butoxide by-product can easily deprotonate this position, forming an enolate species. To finish the reaction and neutralize the molecule, we then perform an acidic workup, adding a proton to the enolate and yielding the final neutral product, which actually resembles an earlier intermediate.
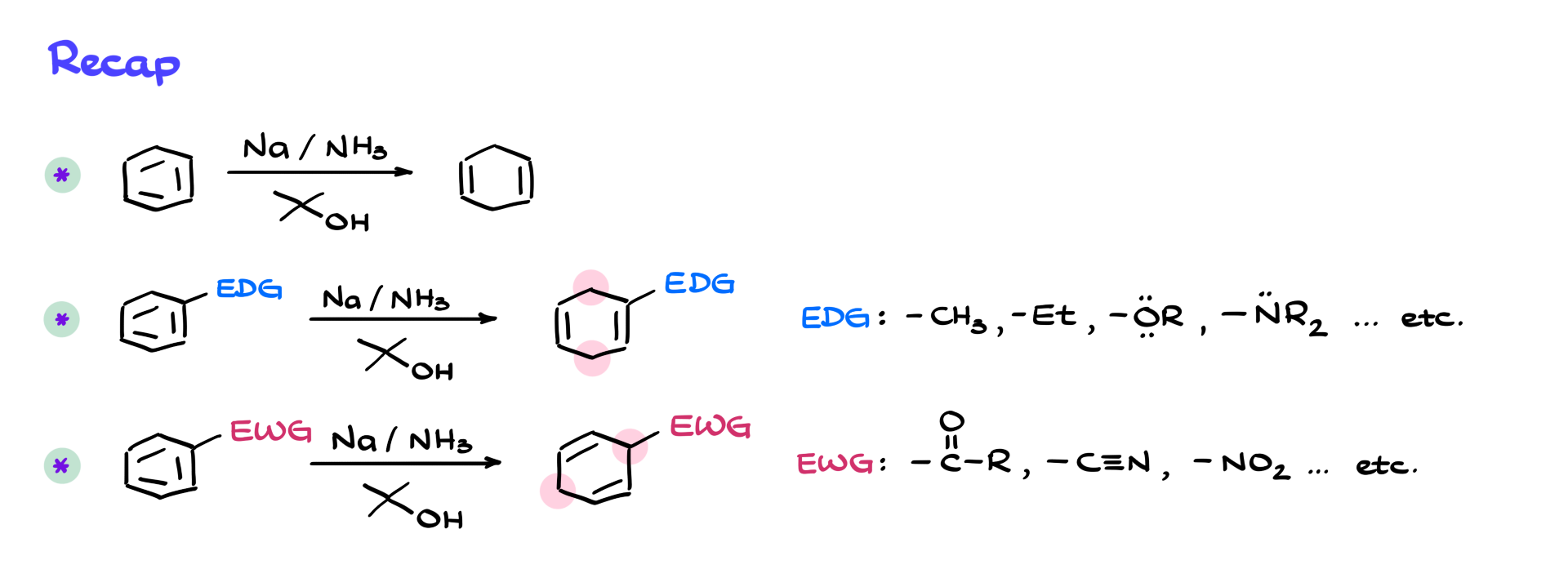
So to recap: If we reduce benzene under Birch conditions, we get 1,4-cyclohexadiene. If we have an electron-donating group, the double bonds end up adjacent to it, and the sp³-hybridized atoms are away from it. Typical donating groups include alkyls, ethers, and amines. With electron-withdrawing groups—like carbonyls, nitriles, or nitro groups—we get the opposite pattern: the sp³-hybridized atoms end up next to the withdrawing group, because that’s where the negative charge sits during the reaction.
Complex Examples of the Birch Reduction
Now what if we have multiple groups? Say a carbonyl (withdrawing) and a methoxy (donating)? We prioritize the position of the electron-withdrawing group, aligning the sp³ hybridized atoms accordingly. After the acidic workup, we get the product that reflects that selectivity.

Another example: a nitrile (withdrawing) and an amine (donating). Again, the withdrawing group dominates, so we place the sp³ hybridized atoms next to it.

One of my favorite examples is the Birch reduction of naphthalene. It has two aromatic rings, so we essentially apply the Birch reduction mechanism twice. In the first reduction, we stabilize the negative charge through resonance with the second ring, so we target benzylic positions for sp³ hybridization. After that, we treat the resulting alkyl groups as electron-donating and adjust accordingly during the second round of reduction, giving us a pretty unusual and complex product.

Birch Alkylation
Now remember: whenever we use electron-withdrawing groups, we follow the Birch reduction with an acidic workup to neutralize the product. But what if we skip that and instead react with an electrophile? That’s where Birch alkylation comes in.
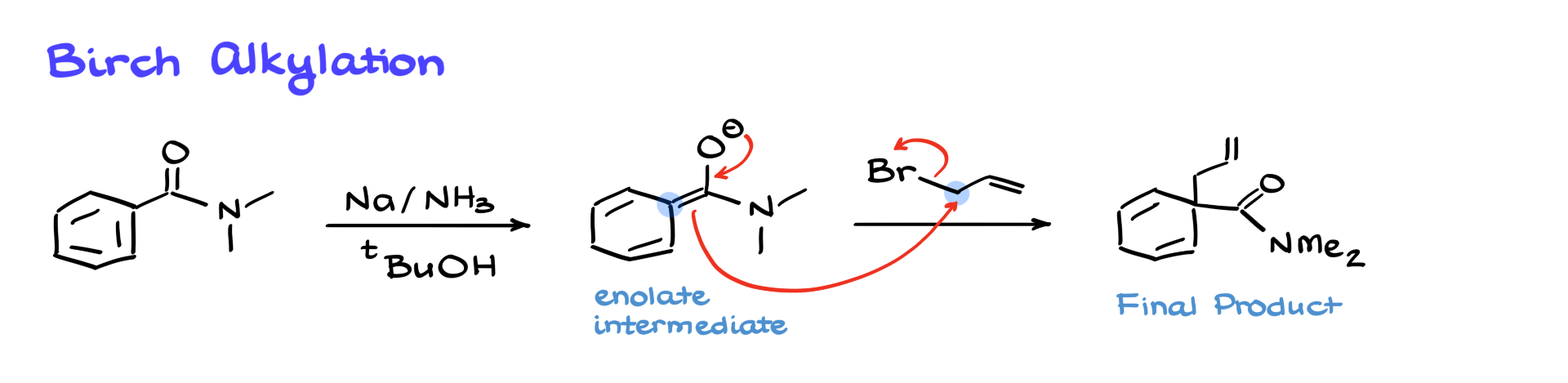
Let’s take an amide and perform a Birch reduction. Instead of protonating the resulting enolate, we introduce an electrophile like allyl bromide. The enolate does a substitution (an SN2 reaction) forming a new bond at the α-position to the former carbonyl, giving us a substituted cyclohexadiene.
This opens up all kinds of synthetic possibilities for constructing highly functionalized six-membered rings with double bonds in unique positions.
Now, I’ve got a challenge for you. Let’s say we perform a Birch reduction on a particular molecule and then react the intermediate with dichloroethane instead of doing an acidic workup. The product ends up being something you might not expect. Can you propose a reasonable mechanism that explains how this transformation occurs? Let me know what you think in the comments.
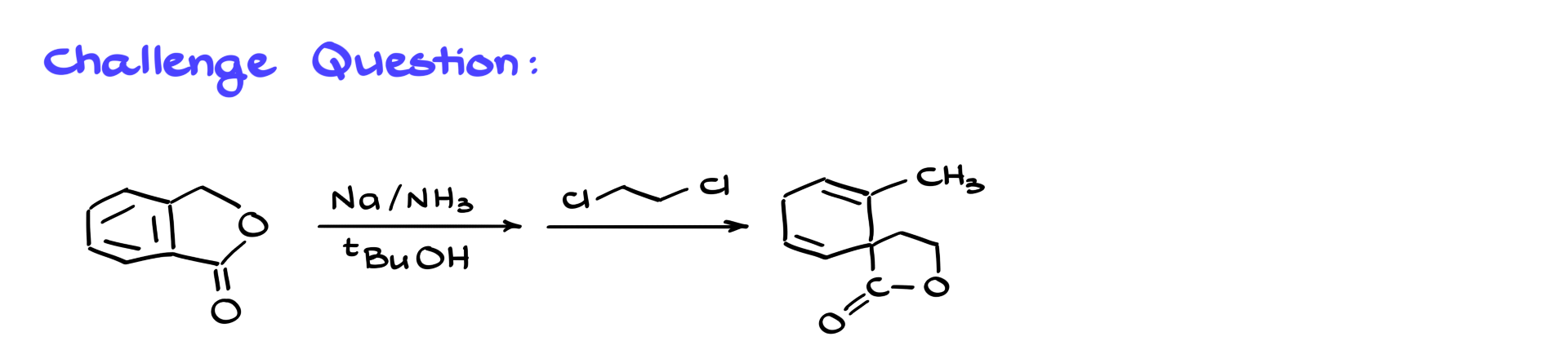